the Creative Commons Attribution 4.0 License.
the Creative Commons Attribution 4.0 License.
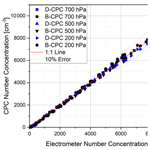
A new working fluid for condensation particle counters for use in sensitive working environments
Oliver F. Bischof
Benedikt Fischer
Marcel Berg
Jannik Schmitt
Gerhard Steiner
Lothar Keck
Andreas Petzold
The working fluid of a condensation particle counter (CPC) is one of its essential characteristics. Butanol is commonly used as the working fluid in alcohol-based CPCs due to its proven performance for various applications and for a wide range of working conditions over the past almost 5 decades. At the same time, butanol has several disadvantages, including its strong and unpleasant odour, negative effects when inhaled over prolonged periods and flammability, making it troublesome to use in places with strict safety regulations. In this work, we propose addressing these negative issues by replacing butanol with dimethyl sulfoxide (DMSO), a substance that has not been used as a CPC working fluid to date. DMSO is an odourless, non-flammable and non-toxic substance that is easily accessible and inexpensive. During thorough experiments, this new substitute working fluid has proven to be stable with respect to its performance for CPCs and storage. We show that DMSO behaves in an equivalent manner to butanol when used to operate a CPC in terms of the instrument's counting efficiency, D50 cut-off diameter (the diameter at which 50 % of the particles are counted with respect to a reference) and concentration linearity. In addition, we have demonstrated this for operating pressures ranging from ambient pressure down to 200 hPa without the need for any safety precautions. Our new working fluid was tested on three CPC units representing two different models, almost always in side-by-side measurements. So far, we have achieved the best results with operating temperatures of 40 ∘C for the CPC's saturator and 5 ∘C for its condenser. To address a less desirable property of DMSO, it could be mixed with a slight amount of water to decrease its freezing point significantly. When mixed accordingly, the substance remains a liquid, even in work environments with temperatures ≤ −10 ∘C, without any impact on the CPC's counting efficiency performance.
- Article
(2221 KB) - Full-text XML
- BibTeX
- EndNote
Aerosol science has come more into focus in recent years. This has been driven by the pandemic (Somsen et al., 2020) and rising awareness of the adverse effects of aerosol particles on aspects such as climate change, air quality, public health and these aspects' interrelations (von Schneidemesser et al., 2015). Specifically, the monitoring of atmospheric aerosol (McMurry, 2000; Rose et al., 2021), including under extreme environmental conditions like measurements on airborne platforms (Petzold et al., 2013), exhaust aerosol from various sources (Giechaskiel et al., 2009; Petzold et al., 2011; Bischof et al., 2020), indoor aerosol (Salimifard et al., 2020) and airborne viruses, is a current key application of condensation particle counters (CPCs).
A CPC can measure the aerosol particle number concentration by increasing the size of nanometre-sized particles in a supersaturated environment to optically detectable droplets. In short, the measurement principle of a common CPC can be expressed in three process steps:
-
saturation, by which vapour is formed from a working fluid inside the flow path of the aerosol sample;
-
supersaturation of the vapour in presence of the sample flow, so that the aerosol particles subsequently act as condensation nuclei, get activated (non-equilibrium growth) and grow by several orders of magnitude to an optically detectable size; and
-
the detection of individual particles through scattered light that occurs when they pass through an optically focused, incident radiation source. In order to collect the scattered light, a photosensitive element, e.g. a photomultiplier or a photo diode (Bischof, 2022), is typically used.
This basic principle dates back to John Aitken, who is known for his early experiments in which he manually counted particles that had grown due to supersaturation of water vapour (Aitken, 1888). Today, three working fluids are most commonly used, namely n-butyl alcohol (or n-butanol), isopropyl alcohol (2-propanol or isopropanol) and water. For all working fluids, the detection efficiency has been characterised over a specific range of operating pressures (Brock et al., 2000; Bundke et al., 2015; Gallar et al., 2006; Hermann et al., 2007) that demonstrate the applicability of each working fluid for low-pressure-operation CPCs. It should be noted that both butanol and isopropanol have a strong and unpleasant odour as well as adverse effects when inhaled over prolonged periods and that their use is limited by the fact that both are highly flammable liquids. In contrast, water has the advantage of avoiding the health and safety concerns of alcohol. The disadvantages of water are that it has a 3 times higher mass diffusion coefficient (Hering et al., 2005; Mei et al., 2021), which increases the consumption of the working fluid during operation, and there is the possibility for the growth of organisms during prolonged times of inactivity. Furthermore, with water as the operating fluid, the activation of particles can be hindered (e.g. by aerosol properties like water solubility and lipophilicity).
In the past, there have been several attempts to find an optimal working fluid. A theoretical approach for working fluids was demonstrated by Magnusson et al. (2003) including mostly alkanes, organic acids, aromatic compounds and alcohol substances, but sulfuric compounds were not included. Diethylene glycol (DEG) was discovered by Iida et al. (2009) with great application for sub-3 nm particles; however, droplets only grow to very small sizes, so a “booster” CPC is needed. Dibutyl sebecat (DOS/DEHS) and other substances were tried by Kupper et al. (2020), with the advantage of not being classified as hazardous by the international Globally Harmonized System (GHS), but these were only applicable for high temperatures (above 100 ∘C) in the CPC saturator.
Our search for a new non-toxic, non-flammable and odourless working fluid was motivated by the requirements of aviation authorities that severely restrict the use of instrumentation on passenger aircraft. The European “In-Service Aircraft for a Global Observing System” (IAGOS; http://www.iagos.org, last access: 2 March 2023) research infrastructure (Petzold et al., 2015) has been created to monitor all essential climate variables of the atmosphere, including aerosol particles (Bojinski et al., 2014). The monitoring takes place through regular and global-scale measurements conducted aboard a fleet of commercial passenger aircraft equipped with automated scientific instrumentation. The IAGOS aerosol package uses a butanol-based CPC, as described in detail by Bundke et al. (2015), and provides 2 years of measurement aboard the IAGOS Civil Aircraft for the Regular Investigation of the Atmosphere Based on an Instrument Container (CARIBIC) flying laboratory. It obtained special permission from the German Lufthansa airline, and its use included the observation of the Raikoke volcanic ash plume. However, the fact that butanol is a flammable liquid strongly hinders the international certification of this instrument for use aboard passenger aircraft.
The new dimethyl sulfoxide (DMSO; C2H6OS; CAS no. 67-68-5; 99.9 %) working fluid that we introduce in this study is hitherto unknown for use in a CPC. However, it is actually known within the aerosol community, as it is an intermediate step in the well-documented chemical reaction beginning with DMS (dimethyl sulfide). The reaction's final product is sulfate, which serves as cloud condensation nuclei (Fung et al., 2022). The principal benefit of DMSO for use on passenger aircraft is that DMSO does not require any safety regulations according to the GHS classification of chemicals. In keeping with this internationally agreed upon system, it does not have any physical or health hazard characteristics. Therefore, this working fluid does not present an obstacle during the certification process for aviation operation. At the global scale, the effect of using DMSO as the working fluid in a CPC is considered minimal for stations that measure DMSO and DMSO products, as the amount of DMSO released to the environment is far less than natural production.
This study is part of the ongoing development of the IAGOS instrumentation package, in response to these flight safety aspects. For the next generation of the IAGOS aerosol package, we will operate two butanol condensation particle counters (model 5411 Sky-CPC; GRIMM Aerosol Technik, Ainring, Germany), which is a variant of the GRIMM model 5410 CPC that uses aviation-grade components and materials, alongside an optical particle counter (OPC; model 1.129, GRIMM). One CPC is dedicated to measuring the total aerosol concentration and the other is equipped with a thermodenuder operated at 250 ∘C upstream to measure only the non-volatile fraction of aerosol particles.
Working fluid characteristics
A working fluid's physical characteristics are important for CPC performance. One governing parameter is the maximum saturation ratio which can be achieved in a specific CPC design. Our first objective was to find a substance with similar physical–chemical properties to butanol or water, which are widely used as working fluids in CPCs. The second motivation was to address any safety restrictions that make the working fluid suitable for sensitive working environments, such as hospitals, schools, public spaces, industrial manufacturing and, specifically, commercial passenger aircraft. DMSO was eventually identified as a candidate substance based on the authors' experience with organic solvents. Besides the advantages of not having any physical or health hazard characteristics, DSMO is also soluble in water. Therefore, the relatively high freezing point of DMSO of 18 ∘C can be adjusted to −100 ∘C (or even below) by simply adding defined amounts of water (Havemeyer, 1966).
The supersaturation of the working fluid vapour determines the smallest possible particle size that will be activated to grow. For pure liquids, this ratio can be expressed through the Kelvin equation (Hinds, 1999; Thomson, 1871):
Here, the following parameters are used: the surface tension of the liquid (σ), the solvent's molecular weight (M), the density (δ), the general gas constant (R), the absolute temperature (T) and the Kelvin diameter (dk). For DMSO, a surface tension of 0.043 N m−1, a molecular weight of 0.078 kg mol−1 and the liquid density of 1100 kg m−3 are used (CRC Handbook; Rumble, 2022).
The Kelvin diameter is the droplet diameter at saturation rate , at which the droplet is in equilibrium and neither starts to grow nor shrink due to condensation processes or evaporation.
When a soluble nucleus is considered, the particle growth can be initiated by a mere percentage needed for a pure liquid. A given mass of a salt reduces the given vapour pressure at the droplet surface. The relationship between the Kelvin ratio and the particle size with dissolved matter is given by Hinds (1999):
where m is the mass of the dissolve material and Ms the molecular weight of the dissolved substance. The constant i is the number of molecules the salt will dissolved into and dp is the droplet diameter. This ratio can then be solved for a particle size when the supersaturation ratio is known. To calculate the saturation vapour pressure, the empirical Antoine equations can be used (Antoine, 1888):
Here, a, b and c are component-specific constants, p is the vapour pressure and T the temperature. Solving the Antoine equation for two temperatures and taking the ratio of the two results yields the supersaturation for this calculated temperature difference. For DMSO, the component-specific constants are a = 5.23, b = 2239.2 and c = −29.2 (Domalski and Hearing, 1996).
To understand the influence of the working fluid on the activation process inside a CPC, the process can be summarised as follows. The vapour pressure above the curved particle surface is described by the Kelvin–Köhler equation (Thomson, 1871), as visualised in Fig. 1 and calculated using Eq. (2). Here, the saturation ratio is defined by the ratio of the actual vapour pressure to the vapour pressure above a flat surface at a given temperature. Particles smaller than the critical diameter grow or shrink in equilibrium with the vapour in their vicinity. If the critical saturation ratio is exceeded in the particle vicinity, the particle will grow past the critical diameter. If the saturation ratio is above one (i.e. supersaturation), the particles will experience non-equilibrium growth. This process is known as particle activation. The critical supersaturation depends on the initial size of the aerosol particles and will be higher for smaller particles (Hinds, 1999). As shown in Fig. 1, the saturation ratio necessary to activate a sodium chloride particle of an initial size of dp = 13 nm is at 1.012 for DMSO, 1.007 for butanol and 1.002 for water. The necessary supersaturation for activation increases with decreasing particle size. For example, for DMSO, particles with diameters of 6 and 3 nm require saturation ratios of 1.037 and 1.078, respectively.
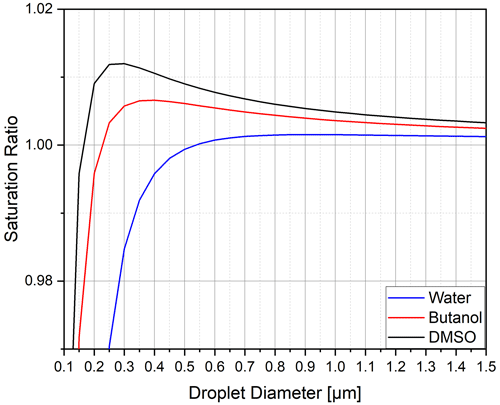
Figure 1Saturation ratio of three working fluids as a function of the droplet diameter for a sodium chloride particle with an initial diameter of 13 nm (Hinds, 1999).
As can be seen in Fig. 2 and as calculated with Eq. (3), the saturation vapour pressure of DMSO is far below that of butanol, although with a similar slope of 0.031, while the slope is 0.026 for water. This indicates that the same supersaturations could be achieved with the same temperature difference between the saturator and condenser of CPCs, although with a much lower consumption of the working fluid. For DMSO, we achieved a supersaturation of about 10 % (saturation ratio of 1.1) with a saturator temperature of 40 ∘C and a temperature drop to 5 ∘C in the condenser part of the CPC used. We are not certain if the signal is generated by droplets or solid crystals, as the freezing point of DMSO is below the condenser temperature.
In the experimental set-up shown in Fig. 3, the sample-line pressure is regulated via the flow mass balance using mass flow controllers (MFCs). The test aerosol is size classified by a differential mobility analyser (DMA; model M-DMA 55-U, GRIMM) while a Faraday cup electrometer (FCE; model 5.705, GRIMM) serves as a reference instrument for the particle concentration. There are two Sky-CPC 5.411 instruments designated B-CPC and D-CPC to indicate the use of butanol and DMSO as the respective operating fluids. In parallel, a TSI® CPC 3772-CEN (TSI Incorporated, Shoreview, MN, USA) that was also operated with DMSO was investigated as well. A more detailed description of the experimental set-up is provided in prior studies (Bundke et al., 2015; Bischof, 2022). To provide a steady and constant particle production from an NaCl–water solution, a continuous output atomiser (COA; model 3076, TSI Incorporated) was used (Liu and Pui, 1975). After the nebulised aerosol flow passes through a diffusion dryer tube, it moves through an aerosol neutraliser equipped with a radioactive Am-241 source. Subsequently, a monodisperse aerosol flow is generated utilising a Vienna-type differential mobility analyser (DMA; model M-DMA 55-U, GRIMM). This monodisperse aerosol is guided to the low-pressure zone by passing through a critical orifice. The aerosol flow is then diluted in a controlled manner within the mixing chamber. The mixing chamber is also used as a buffer volume to smoothen the pressure oscillation generated by the mass balance. The complete test set-up is operated by a LabVIEW™ (National Instruments Corp., Austin, TX, USA) program that was custom-made in our laboratory. Here, several mass flow controllers function as controlling elements with a proportional–integral–derivative (PID) controller approach. Additionally, the relative humidity (RH) can be actively controlled by adding a stable humidified airflow into the mixing chamber. With the added humidified airflow, the relative humidity inside the mixing chamber is limited to approximately 30 % RH. After passing the mixing chamber, the aerosol flow is provided to the measuring instruments along a common sampling line. An individual isokinetic, isoaxial sample inlet in the centre of the sample line guides the aerosol flow to each device.
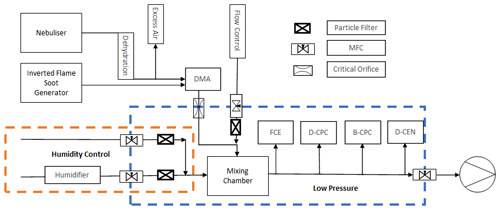
Figure 3Flow schematic of the laboratory set-up for the low-pressure characterisation with two aerosol sources.
During our experiments, the DMA was operated stepwise for 30 s periods to avoid time delays and undefined transition states of aerosol characteristics in the different instruments. Each voltage level corresponds to a different particle size, starting at an upper limit of 140 nm and going down to 2.5 nm in diameter. To avoid transition effects and to achieve an equally distributed aerosol inside all measuring instruments, the first 15 s for each particle size setting of the DMA was excluded from the dataset used for the final analysis.
An inverted flame soot generator (Argonaut Scientific Corp., Edmonton, AB, Canada) was used as a second aerosol source. For stable performance, the soot generator was operated with an oxidation-air-to-propane ratio of 7.5 L min−1 air to 0.0625 L min−1 propane. Previous studies have determined that this flow ratio ensures stable aerosol production with low-organic-carbon soot (Bischof et al., 2020; Kazemimanesh et al., 2018).
As mentioned earlier, the atomiser was used to nebulise salt solutions. Sodium chloride (NaCl) and ammonium sulfate were both used for this purpose. Additionally, fresh combustion soot was measured. In Fig. 4, the corresponding size distributions for these aerosol types are shown. The DMA settings were chosen to provide the greatest possible size resolution at the smallest particle sizes near the range of the expected cut-off diameters. This resulted in a maximum particle mobility size of 138 nm on the upper end for the salts and for the soot. The complete particle size distribution for the soot was taken from Weber et al. (2022).
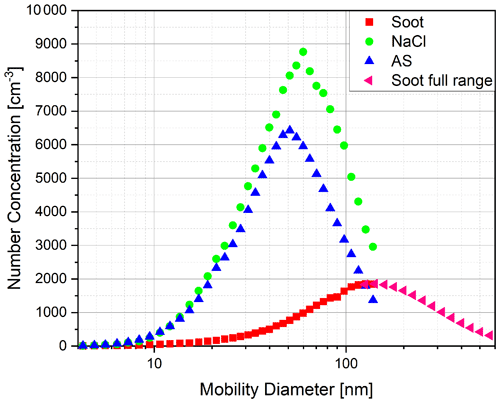
Figure 4Particle size distributions of ammonium sulfate (AS), sodium chloride (NaCl) and fresh combustion soot as measured by the combination of DMA and electrometer.
Data analysis procedure
An important aspect of measuring nanometre-sized particles with the DMA technique is the misclassification of larger, multiply charged particles leaving the DMA with the same mobility as the singly charged particles. This effect leads to a notable difference in the counting rate between a CPC and an aerosol electrometer. To address this artefact, the correction procedure introduced by Bundke et al. (2015) and later adapted by Bischof (2022) was used.
Multiple-charge correction is calculated with a correction scheme that is based on measured size distribution from the CPC and the electrometer in accordance with the DMA theory by Bundke et al. (2015). In order to calculate the counting efficiency ratio, the electrometer and CPC data need corrections or inversions that are displayed in Fig. 5.
The Sky-CPC 5.411 reports an internal diagnosis of the particle growth which is displayed as the C1 C0 value. The C1 C0 reports the ratio of counts that have a higher and a lower detection threshold, which is used as CPC internal check for sufficient particle growth (described in Fig. 6). A value below one indicates that the particles give a lower scattering signal due to a smaller particle size or due to overall light scattering behaviour. Only particles reaching and exceeding both signal threshold levels are counted. The C1 C0 correction is implemented by simply dividing the reported number concentration through the C1 C0 value. In Fig. 6, signals of different droplet sizes are illustrated, along with the detector thresholds C0 and C1. By dividing the reported number concentration by C1 C0, the CPC counts all particles that reach the first detector threshold. DMSO droplets smaller than 2.5 µm will not be counted.
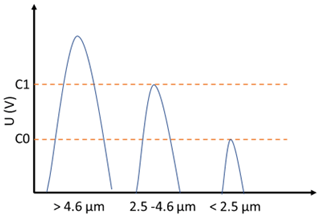
Figure 6Illustration of the pulse height of DMSO droplets of different sizes with corresponding detector threshold levels.
The very need for this correction is due to the low vapour pressure of DMSO. If less material is available for condensation growth, the droplets cannot grow. The diameters of the DMSO droplets that correspond to the signal heights of C0 and C1 are 2.5–2.9 and 4.0–4.6 µm for the different respective thresholds. The values were estimated from the signal heights of latex test particles, a model for scattered light intensities as a function of particle diameter for the GRIMM measuring cell, assuming spherical homogeneous particles, a wavelength of 660 nm, linear polarisation, and refractive indexes of 1.59+0.0i for latex and 1.47+0.0i for DMSO.
To parameterise the efficiency curves, an exponential fit function introduced by Wiedensohler et al. (2018) was applied:
Here, η is the counting efficiency; Dp is the particle size; and A, B and C are fitting parameters calculated using a multiparameter fit (Banse et al., 2001; Wiedensohler et al., 1997), where C corresponds to the D50 cut-off diameter (the diameter at which 50 % of the particles are counted with respect to a reference).
The fitted function was then used to robustly calculate the efficiency for the cut-off of D90.
During initial experiments, we used DMSO from a bottle of our laboratory stock that was first opened 2 years prior to these experiments. We started by completely drying a Sky-CPC 5411. Prior to filling it with DMSO, we performed a test run in the measurement set-up, and the dry CPC reported zero counts. The instrument wick was then wetted with DMSO by the refilling valve, and it worked as intended. The CPC internal fluid controls operated as they do with butanol. We operated this unit, which we refer to as the D-CPC hereafter, with the same parameters as the structurally identical B-CPC that was also included in the experimental set-up (Fig. 3). For the first run, we applied a particle filter to the inlet to check for homogeneous nucleation. No particle counts were observed during zero-particle air measurements, independent of instrument temperatures, humidity or pressure levels. As we started aerosol measurements with pressure levels below 700 hPa, the C1 C0 value decreased to 0.9, and it dropped further down as soon the pressure decreased. As a consequence, we increased the saturator temperature from 36 to 40 ∘C and decreased the condenser temperature from 10 to 5 ∘C. With this procedure, the C1 C0 value stayed at 1 down to a pressure level of 250 hPa and only decreased slightly to 0.95 at 200 hPa. All values given as a number concentration from the D-CPC are corrected by dividing by the C1 C0 value to report all particles at the lower counting threshold, as explained previously. The initial results were obtained with ammonium sulfate particles. The D50 cut-off diameter is used as the primary criterion, as it describes the particle size where only 50 % of the particles are detected compared to a reference method. Figure 7 shows that the D-CPC has an identical D50 cut-off diameter to the B-CPC.
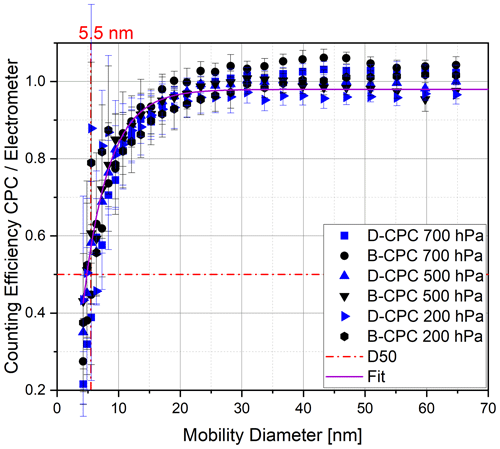
Figure 7Counting efficiency curves of the Sky-CPC 5.411 operated with butanol (B-CPC) and DMSO (D-CPC) at different operation pressures using ammonium sulfate particles.
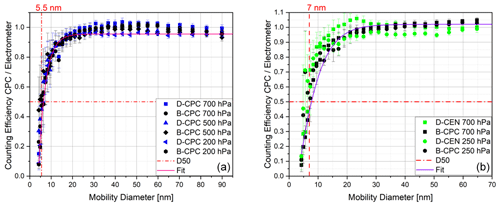
Figure 8Counting efficiency curves for sodium chloride particles with respect to the electrometer reference instrument and corrected for the multiple charge for the Sky-CPC 5.411 (a) and counting efficiency curves for sodium chloride particles with respect to the electrometer reference instrument for the CPC 3772-CEN (b); both instrument types were operated at different operating pressures using butanol (B-CPC) or DMSO (D-CPC, D-CEN) as a working fluid.
As second step, we tested DMSO in a CPC of a different kind. For the CPC 3772-CEN, the main operating principle is the same, but there are slight differences compared with the Sky-CPC. The Sky-CPC has a horizontal wick and a continuous draining of condensed water–butanol mixture located downstream of the saturator working fluid reservoir. The 3772-CEN has a vertical wick that condensed water may flow through before reaching the fluid reservoir. This different design will explain some differences that we observed during the long-term experiments described later on. To start with, we dried the 3772-CEN for over 2 d until no particle counts were reported. We then added the DMSO. We changed the temperature parameters to be equivalent to the D-CPC with 40 ∘C at the saturator and 5 ∘C at the condenser. Therefore, the 3772-CEN was not operated as intended by the European Committee for Standardisation (CEN). In order to highlight this, we will refer to the 3772-CEN operated with DMSO as the D-CEN. As shown in Fig. 8, the counting efficiencies for all CPCs do not significantly differ from each other, regardless of the instrument and applied pressure.
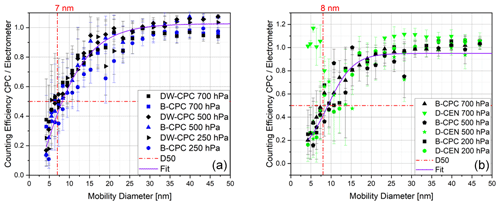
Figure 9Counting efficiency curves with respect to the electrometer reference instrument and corrected for multiple charges at different operating pressures using fresh combustion soot particles for (a) the Sky-CPC 5.411 operated with butanol (B-CPC) and a mixture of DMSO and water (DW-CPC) and (b) the Sky-CPC 5.411 operated with butanol (B-CPC) and the CPC 3772-CEN operated with DMSO (D-CEN).
To test another particle type, we chose fresh combustion soot aerosol, as it is a non-soluble substance and is not hydrophilic. As in the previous experiments, the counting efficiencies for the different CPCs do not differ (Fig. 9).
We repeated all experiments using a mixture of 10 % volumetric water to 90 % DMSO in the Sky-CPC 5.411 (points labelled as DW-CPC in Fig. 9). This mixture does not influence the measurement performance. We observed that the C1 C0 value decreased to below 0.2 for about 1 min as soon as we reached pressure levels below 250 hPa, indicating that water is evaporating and influencing the particle growth. After a span of 1 min, the C1 C0 value rapidly increased to over 0.98. Using this mixture, the lower range of operating conditions is extended to −10 ∘C.
The counting efficiency of the D-CEN for soot particles at 700 hPa exhibits a sudden increase at around 5 nm, as shown in Fig. 9b. This phenomenon needs further investigation, as it could not be explained.
We tested DMSO in our measurement set-up under various conditions. For example, we increased the inline RH to 30 %, although we normally measure at RH below 5 %. Even then, the counting efficiencies as well as the overall behaviour did not change. The instrument cut-off diameters for D50 and D90 do not differ significantly from each other, regardless of particle type, operating fluid or pressure level. In Table 1, the fitting parameters and the derived values for the D90 cut-off diameter are reported for the lowest pressure level studied (either 200 or 250 hPa, depending on the experiment). The significant parameters for the high of the plateau (parameter A; ±0.05) and the cut-off diameter D50 (parameter C; ±1.5) did not change significantly at different pressure levels (200–700 hPa) or humidity levels (0 %–30 % RH).
Table 1Coefficients of the exponential function of the counting efficiency curves and the D90 cut-off diameter (at either 200 or 250 hPa) for different particle types for the Sky-CPC 5.411 operated with butanol (B-CPC) and DMSO (D-CPC) or with a DMSO–water mixture (DW-CPC) and for the CPC 3772-CEN operated with DMSO (D-CEN).
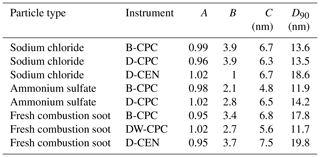
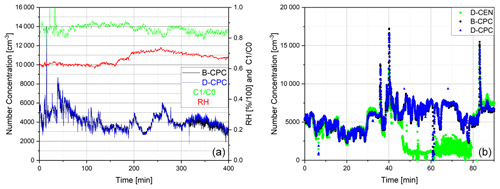
Figure 10Ambient air with relative humidity and C1 C0 values displayed as measured by (a) the Sky-CPC 5.411 operated with butanol (B-CPC) and DMSO (D-CPC) and (b) the B-CPC, D-CPC and CPC 3772-CEN operated with DMSO (D-CEN). D-CEN needed manual extraction of condensed water, which caused the number concentration to drop (see discussion in text).
After characterisation in the lab, we conducted several measurements of outside air at the campus of Forschungszentrum Jülich. The longest measurement lasted 5 consecutive days, and both the D-CPC and the B-CPC measured the same particle concentrations (Fig. 10a). We observed that the 3772-CEN had issues during the measurements caused by DMSO. Here, the valves for draining and priming became blocked by a swollen gasket. First of all, we needed to extract excess fluid from the device manually. As the overall ambient humidity ranged between 60 % and 80 %, it is certain that water condensed at the condenser and dropped into the wick of the 3772-CEN, weakening its activation efficiency. This is seen in Fig. 10b, where the D-CEN trace is lower than the B-CPC trace starting around minute 45. Because DMSO appears to affect the drain and priming valve gaskets, the instrument needed to be manually drained (minute 60 to minute 80 in Fig. 10b). After that, the 3772-CEN returned to the same concentration levels as the B-CPC.
For the ambient aerosol measurement, we recorded the consumption rate: the Sky-CPC was filled with 5 mL of DMSO, whereas the second Sky-CPC was filled with 20 mL of butanol. We ran the measurements overnight. The B-CPC ran dry in the late morning, as expected, and was refilled, whereas the D-CPC was still running and counting. For the first set of ambient measurements, the C1 C0 value of the D-CPC stayed at 1 and decreased slowly until it oscillated around 0.8. In a second approach, we added a water–DMSO mixture, and the C1 C0 value decreased towards 0.6 before slowly going up again to 0.8. In any case, the D-CPC number concentration was corrected with the simultaneously logged data of the C1 C0 value, which were around 0.8 (as seen in Fig. 10).
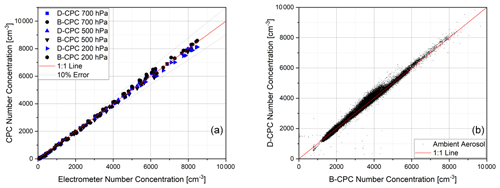
Figure 11(a) Comparison of the concentration linearity of two Sky-CPC 5.411 units to the electrometer reference at different pressure levels for ammonium sulfate and (b) comparison of the correlation between B-CPC and D-CPC on ambient air.
Figure 11 demonstrates that the measurements made using DMSO as the working fluid are not distinguishable from the measurements performed with butanol. The overall linearity for the new working fluid is shown in Fig. 11. The slope of the linear correlation is 1.01 ± 0.02 (r2 > 0.99) for laboratory measurements as well as ambient air measurements. For atmospheric measurements, we used the B-CPC as the well-known reference.
As already mentioned, the CPC 3772-CEN needed manual draining and refilling, and the Sky-CPC 5.411 needed manual refilling. This is because rubber parts soak themselves with DMSO and swell; therefore, they increase in size. As a result, the O-rings expanded inside the valves for refilling and could no longer perform as intended, although it took several days to weeks before the valves stopped working. In order to address this issue, we exchanged those parts with silicon O-rings. We operated the instruments for at least 3 months with the new working fluid and, apart from the valves, no essential parts were affected, as the device kept working. We repeated the process using DMSO as the operating fluid in a third Sky-CPC 5.411; this unit performed the same as the D-CPC in the first experiments, including agreeing well with the B-CPC and the swollen O-ring. As can be in Fig. 12, the O-ring, as well as the stamp of the valve, increased by about 1 mm in size.
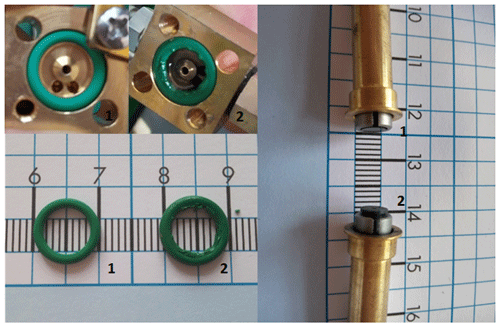
Figure 12Photographs of the CPC O-ring (left panel, top and bottom) and the stem of the liquid supply valve (right panel). The label (1) indicates that the operating fluid was butanol and (2) indicates that the operating fluid was DMSO.
With this increase in size, the sealing ring blocked the stamp and prevented the further flow of liquid to the wick. The continuous pump for draining in the Sky-CPC 5.411 was still working as intended.
Cross-sensitivities between DMSO and several reactive atmospheric substances were not tested. We measured atmospheric aerosol for several weeks using instruments with DMSO as the operating fluid. We did not observe any significant shift in measured particle number concentrations between the reference B-CPC and the D-CPC. However, higher ozone or nitrogen oxide concentrations could trigger secondary organic aerosol activation within the instrument using DMSO.
In the CPC 3772-CEN, one of critical orifices in the supply line of the working fluid started leaking and had to be replaced. It is not clear that this issue can be attributed to the use of DSMO, as the instrument's maintenance state or operational errors could also have been factors.
DMSO can introduce chemicals into the body by transporting dissolved chemicals through the skin. Moreover, bacteria can convert DMSO into dimethyl sulfide. Therefore, DMSO should not be disposed of into the sewer (Tucker, 2014).
Another precaution that should be mentioned here is that DMSO should not be heated to temperatures of over 150 ∘C, as it will lose its thermal stability at the aforementioned threshold. When exceeding 150 ∘C, DMSO slowly starts to degrade into its by-products, which may have different characteristics.
From an instrument manufacturer's point of view, DMSO seems to be an interesting alternative working fluid to the commonly used butanol. Furthermore, at first glance, the modifications required for the correct and safe operation of a GRIMM 5411 Sky-CPC appear to be relatively straightforward. However, as demonstrated in the experiments shown here, there is a need to adapt the CPC design to the specific properties of DMSO, specifically material durability. Furthermore, the detailed condensation process currently remains an open question that needs to be addressed in future studies in order to adapt and optimise the particle detection in upcoming CPC models.
Of equal importance, the benefits of using DMSO as a substitute working fluid by far exceed the effort needed to prepare a CPC for its use (from a user or an instrument manufacturer's perspective). In TSI's most recent generation of butanol CPCs, there are only few places where DMSO comes into contact with materials that it could degrade or swell quickly. In practice, this means that those O-rings and gaskets, which are by default made of polycarbonate (PC), polyvinylidene fluoride (PVDF; like Kynar®) or polyvinyl chloride (PVC), will need to be exchanged for silicone equivalents. While this is not technically difficult, the exact replacement should be assessed and approved separately for every CPC generation and model by the manufacturer. Furthermore, all modifications will need to be tested over much longer periods of time than the brief test period used in this work, as components such as the instrument optics might only be impacted after several weeks or months of exposure to DMSO. Of course, this would change with the introduction of a next generation of alcohol-based CPCs specifically designed for use with DMSO.
In this work, we have introduced dimethyl sulfoxide (DMSO) as an appropriate new substance to replace butanol as the working fluid in alcohol-based condensation particle counters (CPC). DMSO is an odourless, non-flammable and non-toxic substance. We would like to emphasise that DMSO overcomes the notable health and safety concerns that arise when using butanol in sensitive working environments and that this fact alone makes it a desirable candidate for aircraft operation. Secondary benefits include the lower consumption when operating CPCs and its lower cost.
In our experiments, we have demonstrated that the two different CPC models that we tested continued to operate as expected under several operational conditions. When changing the operation pressure from ambient level down to 200 hPa, which corresponds to the cruising level of passenger aircraft used within the IAGOS research infrastructure, the DMSO-operated CPC units displayed the same counting efficiencies as the butanol-operated CPCs. Even in very humid environments with an RH of around 80 %, the CPC operated with DMSO displayed equivalent performance to a butanol CPC. It should be noted that the CPC 3772-CEN had to be drained frequently at those high levels of humidity, and the Sky-CPC 5.411 had to be corrected using its internally recorded C1 C0 value. Nevertheless, for all pressure levels and particle types investigated, the DMSO-operated CPC displayed essentially identical performance to the equivalent butanol-operated CPC. Further investigations with different temperatures for saturator and condenser are planned.
The introduction of DMSO as the working fluid requires no significant modifications to a CPC's hardware apart from minor changes to its liquid supply valve and a replacement of any gasket that comes into contact with the working fluid. Our initial results also indicate that the lower detection limit of the CPC might be even smaller with the new working fluid, as a higher supersaturation can be achieved. This is due to the fact that DMSO has a far higher flash point than butanol. This will be investigated further in cooperation with CPC manufacturers by using a custom-designed saturator block, heating and reservoir.
Data are available at Zenodo: https://doi.org/10.5281/zenodo.8052880 (Weber et al., 2023).
PW and UB conceived of the study. PW performed all instrument maintenance, experiments and data analysis. PW, UB and OFB set up the instruments. UB, PW and BF designed the LabVIEW™ environment of the experimental set-up. MB and JS helped during instrument preparation. LK calculated the droplet size for the threshold detection. PW, OFB, GS, UB and AP contributed to the manuscript and the interpretation of the results.
Oliver F. Bischof is a full-time employee of TSI GmbH, a subsidiary of TSI Incorporated, which has a potential direct or indirect financial interest in the subject matter discussed in the paper. Gerhard Steiner and Lothar Keck are full-time employees of GRIMM Aerosol Technik Ainring GmbH & Co. KG, which also has a potential direct or indirect financial interest in the subject matter discussed in the paper. The other authors declare that they have no conflict of interest.
Weber, P. and Bundke, U.: Verfahren und Vorrichtung zur Vergrößerung von Aerosolpartikeln, German patent PT 0.3349/pa, filed 23 November 2022.
Publisher’s note: Copernicus Publications remains neutral with regard to jurisdictional claims in published maps and institutional affiliations.
This work would not have been possible without the continuous encouragement and support of Astrid Kiendler-Scharr, director of the Institute of Energy and Climate Research 8 at Forschungszentrum Jülich, who passed away unexpectedly in February 2023. We gratefully dedicate our work to her memory.
Parts of this work were supported by the German Ministry of Research and Education within the framework of the IAGOS-D joint research project (grant agreement no. 01LK1301A) and by the HITEC Graduate School for Energy and Climate at Forschungszentrum Jülich. A special thanks to Jürgen Dornseiffer for providing the very first amounts of liquid that we needed to perform initial tests.
Kynar® is a registered trademark of Arkema Incorporated, LabVIEW is a trademark of National Instruments Corporation, and TSI® is a registered trademark of TSI Incorporated.
This research has been supported by the Bundesministerium für Bildung und Forschung (grant no. 01LK1301A) and the Forschungszentrum Jülich (HITEC).
This paper was edited by Hilkka Timonen and reviewed by two anonymous referees.
Aitken, J.: On the Number of Dust Particles in the Atmosphere1, Nature, 37, 428–430, https://doi.org/10.1038/037428a0, 1888.
Antoine, C.: Tensions des vapeurs: nouvelle relation entre les tensions et les températures, Comptes Rendus des Séances de l'Académie des Sciences, 107, 681–684, 778–780, 836–837, 1888.
Banse, D. F., Esfeld, K., Hermann, M., Sierau, B., and Wiedensohler, A.: Particle counting efficiency of the TSI CPC 3762 for different operating parameters, J. Aerosol Sci., 32, 157–161, https://doi.org/10.1016/S0021-8502(00)00060-4, 2001.
Bischof, O. F.: Application-Specific Calibration of Condensation Particle Counters under Low Pressure Conditions, Dissertation, Verlag des Forschungszentrums Jülich, Energie Umwelt, Band 576, ISBN 978-3-95806-629-8, 2022.
Bischof, O. F., Weber, P., Bundke, U., Petzold, A., and Kiendler-Scharr, A.: Characterization of the Miniaturized Inverted Flame Burner as a Combustion Source to Generate a Nanoparticle Calibration Aerosol, Aerosol, Emiss. Control Sci. Technol., 6, 37–46, https://doi.org/10.1007/s40825-019-00147-w, 2020.
Bojinski, S., Verstraete, M., Peterson, T. C., Richter, C., Simmons, A., and Zemp, M.: The Concept of Essential Climate Variables in Support of Climate Research, Applications, and Policy, B. Am. Meteorol. Soc., 95, 1431–1443, https://doi.org/10.1175/BAMS-D-13-00047.1, 2014.
Brock, C. A., Schröder, F., Kärcher, B., Petzold, A., Busen, R., and Fiebig, M.: Ultrafine particle size distributions measured in aircraft exhaust plumes, J. Geophys. Res.-Atmos., 105, 26555–26567, https://doi.org/10.1029/2000JD900360, 2000.
Bundke, U., Berg, M., Houben, N., Ibrahim, A., Fiebig, M., Tettich, F., Klaus, C., Franke, H., and Petzold, A.: The IAGOS-CORE aerosol package: instrument design, operation and performance for continuous measurement aboard in-service aircraft, Tellus B, 67, 286–302, https://doi.org/10.3402/tellusb.v67.28339, 2015.
Domalski, E. S. and Hearing, E. D.: Heat Capacities and Entropies of Organic Compounds in the Condensed Phase. Volume III, J. Phys. Chem. Ref. Data, 25, 1–525, https://doi.org/10.1063/1.555985, 1996.
Fung, K. M., Heald, C. L., Kroll, J. H., Wang, S., Jo, D. S., Gettelman, A., Lu, Z., Liu, X., Zaveri, R. A., Apel, E. C., Blake, D. R., Jimenez, J.-L., Campuzano-Jost, P., Veres, P. R., Bates, T. S., Shilling, J. E., and Zawadowicz, M.: Exploring dimethyl sulfide (DMS) oxidation and implications for global aerosol radiative forcing, Atmos. Chem. Phys., 22, 1549–1573, https://doi.org/10.5194/acp-22-1549-2022, 2022.
Gallar, C., Brock, C. A., Jimenez, J. L., and Simons, C.: A Variable Supersaturation Condensation Particle Sizer, Aerosol Sci. Tech., 40, 431–436, https://doi.org/10.1080/02786820600643339, 2006.
Giechaskiel, B., Wang, X., Horn, H. G., Spielvogel, J., Gerhart, C., Southgate, J., Jing, L., Kasper, M., Drossinos, Y., and Krasenbrink, A.: Calibration of Condensation Particle Counters for Legislated Vehicle Number Emission Measurements, Aerosol Sci. Tech., 43, 1164–1173, https://doi.org/10.1080/02786820903242029, 2009.
Gutmann, F. and Simmons, L. M.: A Theoretical Basis for the Antoine Vapor Pressure Equation, J. Chem. Phys., 18, 696–697, https://doi.org/10.1063/1.1747730, 1950.
Havemeyer, R. N.: Freezing Point Curve of Dimethyl Sulfoxide – Water Solutions, J. Pharm. Sci., 55, 851–853, https://doi.org/10.1002/jps.2600550822, 1966.
Hering, S. V., Stolzenburg, M. R., Quant, F. R., Oberreit, D. R., and Keady, P. B.: A Laminar-Flow, Water-Based Condensation Particle Counter (WCPC), Aerosol Sci. Tech., 39, 659–672, https://doi.org/10.1080/02786820500182123, 2005.
Hermann, M., Wehner, B., Bischof, O. F., Han, H.-S., Krinke, T., Liu, W. S., Zerrath, A. F., and Wiedensohler, A.: Particle counting efficiencies of new TSI condensation particle counters, J. Aerosol Sci., 38, 674–682, 2007.
Hinds, W. C.: Aerosol Technology: Properties, Behavior, and Measurement of Airborne Particles, Wiley, ISBN 0-471-19410-7, 1999.
Iida, K., Stolzenburg, M. R., and McMurry, P. H.: Effect of Working Fluid on Sub-2 nm Particle Detection with a Laminar Flow Ultrafine Condensation Particle Counter, Aerosol Sci. Tech., 43, 81–96, https://doi.org/10.1080/02786820802488194, 2009.
Kazemimanesh, M., Moallemi, A., Thomson, K., Smallwood, G., Lobo, P., and Olfert, J.: A novel miniature inverted-flame burner for the generation of soot nanoparticles, Aerosol Sci. Tech., 53, 184–195, https://doi.org/10.1080/02786826.2018.1556774, 2018.
Kupper, M., Kraft, M., Boies, A., and Bergmann, A.: High-temperature condensation particle counter using a systematically selected dedicated working fluid for automotive applications, Aerosol Sci. Tech., 54, 381–395, https://doi.org/10.1080/02786826.2019.1702920, 2020.
Liu, B. Y. H. and Pui, D. Y. H.: On the performance of the electrical aerosol analyzer, J. Aerosol Sci., 6, 249–264, https://doi.org/10.1016/0021-8502(75)90093-2, 1975.
Magnusson, L.-E., Koropchak, J., Anisimov, M., Poznjakovskiy, V., and Fernandez de la Mora, J.: Correlations for Vapor Nucleating Critical Embryo Parameters, J. Phys. Chem. Ref. Data, 32, 1387–1409, https://doi.org/10.1063/1.1555590, 2003.
McMurry, P. H.: A review of atmospheric aerosol measurements, Atmos. Environ., 34, 1959–1999, https://doi.org/10.1016/s1352-2310(99)00455-0, 2000.
Mei, F., Spielman, S., Hering, S., Wang, J., Pekour, M. S., Lewis, G., Schmid, B., Tomlinson, J., and Havlicek, M.: Simulation-aided characterization of a versatile water-based condensation particle counter for atmospheric airborne research, Atmos. Meas. Tech., 14, 7329–7340, https://doi.org/10.5194/amt-14-7329-2021, 2021.
Petzold, A., Marsh, R., Johnson, M., Miller, M., Sevcenco, Y., Delhaye, D., Ibrahim, A., Williams, P., Bauer, H., Crayford, A., Bachalo, W. D., and Raper, D.: Evaluation of methods for measuring particulate matter emissions from gas turbines, Environ. Sci. Technol., 45, 3562–3568, https://doi.org/10.1021/es103969v, 2011.
Petzold, A., Formenti, P., Baumgardner, D., Bundke, U., Coe, H., Curtius, J., DeMott, P. J., Flagan, R. C., Fiebig, M., Hudson, J. G., McQuaid, J., Minikin, A., Roberts, G. C., and Wang, J.: In Situ Measurements of Aerosol Particles, in: Airborne Measurements for Environmental Research, Wiley-VCH Verlag GmbH & Co. KGaA, 157–223, https://doi.org/10.1002/9783527653218.ch4, 2013.
Petzold, A., Thouret, V., Gerbig, C., Zahn, A., Brenninkmeijer, C. A. M., Gallagher, M., Hermann, M., Pontaud, M., Ziereis, H., Boulanger, D., Marshall, J., Nédélec, P., Smit, H. G. J., Friess, U., Flaud, J.-M., Wahner, A., Cammas, J.-P., Volz-Thomas, A., and IAGOS TEAM: Global-scale atmosphere monitoring by in-service aircraft – current achievements and future prospects of the European Research Infrastructure IAGOS, Tellus B, 67, 28452, https://doi.org/10.3402/tellusb.v67.28452, 2015.
Rose, C., Collaud Coen, M., Andrews, E., Lin, Y., Bossert, I., Lund Myhre, C., Tuch, T., Wiedensohler, A., Fiebig, M., Aalto, P., Alastuey, A., Alonso-Blanco, E., Andrade, M., Artíñano, B., Arsov, T., Baltensperger, U., Bastian, S., Bath, O., Beukes, J. P., Brem, B. T., Bukowiecki, N., Casquero-Vera, J. A., Conil, S., Eleftheriadis, K., Favez, O., Flentje, H., Gini, M. I., Gómez-Moreno, F. J., Gysel-Beer, M., Hallar, A. G., Kalapov, I., Kalivitis, N., Kasper-Giebl, A., Keywood, M., Kim, J. E., Kim, S.-W., Kristensson, A., Kulmala, M., Lihavainen, H., Lin, N.-H., Lyamani, H., Marinoni, A., Martins Dos Santos, S., Mayol-Bracero, O. L., Meinhardt, F., Merkel, M., Metzger, J.-M., Mihalopoulos, N., Ondracek, J., Pandolfi, M., Pérez, N., Petäjä, T., Petit, J.-E., Picard, D., Pichon, J.-M., Pont, V., Putaud, J.-P., Reisen, F., Sellegri, K., Sharma, S., Schauer, G., Sheridan, P., Sherman, J. P., Schwerin, A., Sohmer, R., Sorribas, M., Sun, J., Tulet, P., Vakkari, V., van Zyl, P. G., Velarde, F., Villani, P., Vratolis, S., Wagner, Z., Wang, S.-H., Weinhold, K., Weller, R., Yela, M., Zdimal, V., and Laj, P.: Seasonality of the particle number concentration and size distribution: a global analysis retrieved from the network of Global Atmosphere Watch (GAW) near-surface observatories, Atmos. Chem. Phys., 21, 17185–17223, https://doi.org/10.5194/acp-21-17185-2021, 2021.
Rumble, J. R. (Ed.): CRC Handbook of Chemistry and Physics, 103rd edn., CRC Press/Taylor & Francis, Boca Raton, https://hbcp.chemnetbase.com/faces/contents/ContentsResults.xhtml (last access: 2 March 2023), 2022.
Salimifard, P., Rim, D., and Freihaut, J. D.: Evaluation of low-cost optical particle counters for monitoring individual indoor aerosol sources, Aerosol Sci. Tech., 54, 217–231, https://doi.org/10.1080/02786826.2019.1697423, 2020.
Somsen, G. A., van Rijn, C. J. M., Kooij, S., Bem, R. A., and Bonn, D.: Measurement of small droplet aerosol concentrations in public spaces using handheld particle counters, Phys. Fluids, 32, 121707, https://doi.org/10.1063/5.0035701, 2020.
Thomson, W.: LX. On the equilibrium of vapour at a curved surface of liquid, The London, Edinburgh, and Dublin Philosophical Magazine and Journal of Science, 42, 448–452, https://doi.org/10.1080/14786447108640606, 1871.
Tucker, S. P.: Development, evaluation and comparison of two independent sampling and analytical methods for ortho-phthalaldehyde vapors and condensation aerosols in air, Anal. Methods-UK, 6, 2592–2607, https://doi.org/10.1039/C3AY42085J, 2014.
von Schneidemesser, E., Monks, P. S., Allan, J. D., Bruhwiler, L., Forster, P., Fowler, D., Lauer, A., Morgan, W. T., Paasonen, P., Righi, M., Sindelarova, K., and Sutton, M. A.: Chemistry and the Linkages between Air Quality and Climate Change, Chem. Rev., 115, 3856–3897, https://doi.org/10.1021/acs.chemrev.5b00089, 2015.
Weber, P., Petzold, A., Bischof, O. F., Fischer, B., Berg, M., Freedman, A., Onasch, T. B., and Bundke, U.: Relative errors in derived multi-wavelength intensive aerosol optical properties using cavity attenuated phase shift single-scattering albedo monitors, a nephelometer, and tricolour absorption photometer measurements, Atmos. Meas. Tech., 15, 3279–3296, https://doi.org/10.5194/amt-15-3279-2022, 2022.
Weber, P., Bischof, O. F., Fischer, B., Berg, M., Schmitt, J., Steiner, G., Keck, L., and Bundke, U.: Dataset: A new working fluid for condensation particle counters for use in sensitive working environments (Version 1), Zenodo [data set], https://doi.org/10.5281/zenodo.8052880, 2023.
Wiedensohler, A., Orsini, D., Covert, D. S., Coffmann, D., Cantrell, W., Havlicek, M., Brechtel, F. J., Russell, L. M., Weber, R. J., Gras, J., Hudson, J. G., and Litchy, M.: Intercomparison Study of the Size-Dependent Counting Efficiency of 26 Condensation Particle Counters, Aerosol Sci. Tech., 27, 224–242, https://doi.org/10.1080/02786829708965469, 1997.
Wiedensohler, A., Wiesner, A., Weinhold, K., Birmili, W., Hermann, M., Merkel, M., Müller, T., Pfeifer, S., Schmidt, A., Tuch, T., Velarde, F., Quincey, P., Seeger, S., and Nowak, A.: Mobility particle size spectrometers: Calibration procedures and measurement uncertainties, Aerosol Sci. Tech., 52, 146–164, https://doi.org/10.1080/02786826.2017.1387229, 2018.